Kinematic, Thermodynamic and Structural Factors Governing the Dispersion of Nanoclays in Polymer Melts¶
Mark D. Wetzel
DuPont, Wilmington, Delaware, 19880Abstract
Polymer nanocomposites offer a potential unique solution
to improve desired physical attributes while maintaining
other incompatible properties. For example,
increasing the mechanical stiffness and strength of ionomers
while preserving toughness and optical performance
may not be possible with conventional, micron-scale fillers.
In order to demonstrate significant property enhancements,
a well-controlled dispersion of nano-scale
fillers in a polymer matrix is required. Melt processing
represents the most economical and flexible route to producing
thermoplastic nanocomposites, but may not
achieve dispersion quality as has been demonstrated by
other methods. This paper describes melt compounding
experiments that help establish kinematic, thermodynamic
and structural factors that govern the dispersion of
layered silicates in ionomers. The results show that the
stress imparted during the melt blending influences dispersion.
Platelet size also influences the extent of exfoliation
in the extruder. However, the thermodynamic
compatibility between the filler surface, organic modifier
and matrix polymer plays a dominant role in the exfoliation
process.
Introduction
In order to demonstrate significant property enhancements,
a well-controlled dispersion of a nano-filler
into a polymer matrix is required. A good dispersion can
be characterized by a particle size distribution approaching
primary particle dimensions distributed uniformly
through the host polymer. For layered silicates, the
process must first break apart and wet large aggregates of
tactoids (cohesive platelet stacks) and then exfoliate
platelets (delaminate) from the tactoids. For a given
shaping and forming process (injection molding, blown
film, extruded film, etc.) an anisotropic nanocomposite
structure will be created with platelets and tactoids aligning
with a shear or extensional flow field. Physical properties
of interest depend on intrinsic matrix and filler
properties, interfacial adhesion between the filler, surface
modifier and polymer, the state of dispersion and particle
orientations induced by processing [1-3].
Melt processing represents an economical and flexible
route for producing thermoplastic nanocomposites.
However, conventional melt compounding systems usually
cannot create dispersions of the same quality as made
with solution or in-situ polymerization methods. Thus, a
conventional melt blending system may represent a tradeoff
between economics and dispersion quality. To date, few, if any academic or commercial publications have
achieved perfect exfoliation of layered silicates in thermoplastics
using conventional batch mixers or extruders.
In compounding experiments, organically modified
layered silicates were dispersed into ethylene copolymer
ionomers using a twin-screw extrusion process. The extent
of exfoliation was examined by microscopy and
through tensile property measurements of injection
molded samples. This work reproduces and extends research
on ionomer / clay nanocomposites conducted by
Paul and associates [4-6]. They investigated the effects
of counter-ion type (Li, Na, Zn) in Surlyn® ethylene copolymers
along with the type of quaternary ammonium
organic modifier on Montmorillonite (MMT) clay.
Kamal and his co-authors reported that the thermodynamic
interactions between the clay, modifier and polymer
have a significant effect on the cohesive force between
platelets by changing the van der Waals forces and the
inter-gallery spacing [1]. Furthermore, they quantified
the stress required in a polymer melt under simple shear
to promote delamination of platelets or separation of child
tactoids from parent particles.
Experimental
A ZSK-18mm intermeshing, co-rotating twin-screw
extruder (Coperion GmbH, Stuttgart, Germany) fitted
with 10 barrels was used to compound ethylene copolymer
ionomers with organically modified layered silicates.
A conventional compounding screw with a 41 L/D was
used with a melting zone followed by one mixing section
comprised of kneading blocks and reverse conveying
elements. DuPont Surlyn® (partially neutralized ethylene/methacrylic
acid copolymer) sodium 8945 (4.5 g/10
min at 190°C melt index) and zinc 9945 (4 g/10 min at
190°C melt index) ionomers were selected based on
Paul’s work [4-6]. Several 2:1 layered silicates, including
organically modified Montmorillonite (MMT: Cloisite®
20A, 15A, 6A and 93A), unmodified MMT (Cloisite
Na+) and synthetic Hectorite (Laponite® RD and OG) and
fluorohectorite (Laponite B) were supplied by Southern
Clay Products, Gonzalez, Texas. Cloisite 93A uses a
more polar methyl, dihydrogenated tallow ammonium
surfactant (designated MH(HT)2 with one methyl group
replaced by one hydrogen) while all other clays were ion
exchanged with a dimethyl, dihydrogenated tallow, quaternary
ammonium moiety (designated M2(HT)2). Clay
types, modifier type, amount exchanged and inter-gallery
spacing measured by wide angle X-ray scattering
(WAXS) are listed in Table 1.
The ingredients were added using two loss-in-weight
feeders, with polymer pellets metered into the first feed
port and clay powders side-fed into barrel #4 into the
molten ionomer for most tests. Several tests fed both
materials in the rear feed port. The total feed rate was
varied from approximately 4.5 to 9 kg/hr (10 to 20 lb/hr)
with a range of powder concentrations from 3 to 10
weight percent clay content. The extruder screw speed
was fixed at 350 RPM for all states, with the exception of
a 9 kg/hr / 700RPM run. Barrel temperatures were fixed
between 160 to 220°C from inlet to the die. In several
tests, 25 wt. % Cloisite 20A masterbatches were prepared
with melt mixing zone barrel temperatures set to 160 and
210°C to emulate high and low stress states, respectively.
The concentrates were then let down to final compositions
in a second extrusion step. Pellet samples were collected,
dried and then molded into ASTM tensile bars
using an Arburg Allrounder 1.5oz injection molding machine
using standard Surlyn drying and operating conditions.
Tensile data were measured using ASTM standard
638D on dry as molded samples. Transmission Electron
Microscopy (TEM) micrographs were made with approximately
90nm thick microtomed cross-sections cut from
the center of the bars, perpendicular to the flow direction.
Discussion of Results
Process Kinematic Effects
Figure 1 shows the shear stress for the sodium and
zinc ionomers plotted as a function of shear rate and temperature.
The curves are model predictions based on measurements
in a capillary rheometer. While the zinc
Surlyn has a higher viscosity than the sodium resin at
160°C, the viscosities are closely matched at higher temperatures
typically experienced in the melt mixing zones
of the extruder. The ZSK-18mm extruder has a high surface
area to free volume providing significant heat transfer
to control melt temperature. Masterbatches containing
25 wt. % MMT were prepared by melt compounding with
the melt mixing zone barrels set to 160 and 220°C to impose
high and low stress states on the sodium ionomer
clay mixtures. The concentrate pellets were then co-fed
with the sodium ionomer in a second extrusion operated
with barrels set to a conventional profile to produce 3, 5
and 7 wt. % MMT nanocomposites.
Figure 2 shows tensile properties of the letdown
compositions as a function of MMT loading and masterbatch
process barrel temperature. Paul showed that the
tensile modulus of MMT nanocomposites is influenced
by the extent of exfoliation as indicated by a measurement
or estimate of an effective aspect ratio [3-6]. The
low temperature states show a clear increase in modulus
over the high temperature states. From the rheology measurements,
the shear stress at the 160°C condition would
be significantly higher than at 210°C. It is likely that
more tactoids would experience the critical stress required
to overcome the cohesive force to exfoliate plate lets or split tactoid fragments. The elongation at break
also changed with masterbatch compounding barrel temperature.
The low temperature condition resulted in a
decrease in the strain at break with MMT loading. For
the high temperature state, the elongations were higher.
Another experiment was conducted to explore the effects
of throughput (Q) and screw speed (N) on MMT
dispersion in the sodium ionomer. Cloisite 20A was metered
into the first feed port or the side stuffer at 3, 5, 7
and 10 wt. % MMT content. Two constant Q/N conditions
were run. Tensile properties of injection molded
samples are shown in Figure 3. Clay fed in the rear of the
extruder experienced the high stress of the melting zone.
This likely changed the extent of exfoliation and the effective
aspect ratio as indicated by the tensile modulus.
For each feed method, the modulus and elongations for
almost all composition states were very similar at the
constant Q/N conditions.
From Figure 1, the shear stress at a screw speed of
700 RPM is slightly higher than at 350 RPM. The energy
dissipation, and hence the melt temperature are anticipated
to be higher at 700 RPM, reducing the average
stress in the channel. The mean residence time at 4.5
kg/hr (10 lb/hr) and 350 RPM is expected to be longer
than at the 9.2 kg/hr (20 lb/hr) and 700 RPM condition [7,
8]. It is likely that the dispersion quality was similar for
the two conditions with the same degree of fill. This result
indicates that a melt compounding process can be
operated in different regimes where a balance of residence
time, stress and melt temperature can be found to
produce the microstructures with similar physical properties.
Thermodynamic and Structural Effects
The choice of MMT surface modifier and the excess
amount of surfactant above the clay cation exchange capacity
(CEC) have significant effects on processing, dispersion
and physical properties. Figure 4 shows the die
hand melt temperature and exit pressure as a function
modifier type and MMT concentration at the 4.5 kg/hr
(10 lb/hr) and 350 RPM condition. Temperature and
pressure increased with loading for Cloisite 20A
[M
2(HT)
2 modifier, CEC 95 milli-equivalents/100gm clay
(meq)]. The temperate and pressure increase with filler
loading was reduced when excess surfactant was applied
(Cloisite 15A, CEC 125 meq, and 6A, CEC 140 meq).
This indicates that the excess modifier may act as a plasticizer.
Excess surfactant likely dispersed in the polymer,
reducing melt viscosity. Thermogravimetric analysis
(TGA) tests on the organically modified MMT and sodium
ionomer nanocomposites are shown in Figure 15.
The TGA in air show that the clays and nanocomposites
are thermally stable up to the process temperatures experienced
in the extrusion tests (typically less than 240°C).
The Cloisite 15A and 6A did show more weight loss, due
to the free excess surfactant. Although is known that quaternary ammonium salts degrade through Hoffman
elimination beginning at 160 to 170°C, the potential degradation
products of the bound and excess surfactant did
not appear to be a primary cause of plasticization (or additional
discoloration of the samples). TEM micrographs
in Figure 8 and 9 show that the Cloisite 20A and 15A
composites with 5 wt. % MMT content had similar micro/nano-structures
consisting of individual exfoliated
platelets and a distribution of tactoids. For this experiment,
the excess surfactant in the 15A clay did not improve
the dispersion quality measurably. The tensile
properties of the Cloisite 20A, 15A and 6A nanocomposites
are shown in Figure 5. The modulus and elongations
were very similar with MMT loading. The excess
M
2(HT)
2 modifier may have improved the extent of exfoliation
(visually less haze) and hence, the effective aspect
ratios slightly. Improved dispersion may have
compensated for the plasticizing effect, especially with
the Cloisite 6A (CEC 140 meq).
Cloisite 93A treated with a more polar modifier
[MH(HT)2, CEC 90 meq] appeared to be thermodynamically
incompatible with the sodium ionomer as indicated
by decreasing process melt temperatures and pressures
shown in Figure 4. Higher filler loading should have
increased melt viscosity. If the exfoliation process produced
a particle size distribution capable of forming a
rheologically percolating network, the melt viscosity
should have increased significantly [1]. The TGA data in
Figure 15 show that the Cloisite 93A clay and the nanocomposite
were thermally stable over the processing temperatures
experienced. The sodium ionomer / 93A
composite was more thermally stable than those using
MMT modified with M
2(HT)
2. The TEM micrographs in
Figure 10 show that the melt process failed to produce a
good dispersion. The micro/nano-structure consisted of a
small number of exfoliated platelets and a dominant population
of large tactoids and agglomerates. This morphology
resulted in little increase in the Young’s modulus
shown in Figure 5. However, Cloisite 93A composites
had a significant increase in the elongation at break from
160 to 240%. Usually, a poor dispersion would not contribute
to a large increase in the elongation (large agglomerate
stress concentrators and flaw sites). This is strong
indication that the MH(HT)
2 modifier was incompatible
with the sodium Surlyn, resulting in poor interfacial adhesion
which, in part, increased the toughness. Furthermore,
the large tactoids could induce cavitation upon
fracture, dissipating energy that could also increase the
toughness [10]. This experiment shows that the thermodynamic
compatibility between filler-modifier-polymer
system components is critical in promoting good dispersion
in the melt blending process and providing sufficient
interfacial adhesion in the nanocomposites resulting in
significant reinforcement [1].
In the next experiment, the zinc ionomer was melt
compounded with the same set of MMT clays. Unmodified MMT (Cloisite Na+) was included in the composition
series. All clays were introduced into the extruder in
the side feed location. Figure 11 shows TEM micrographs
of the zinc ionomer/Cloisite 20A (5 wt. % MMT)
composite. While there is a small population of submicron
particles, the dispersion represents a significant improvement
in extent of exfoliation as compared with the
sodium ionomer. The tensile properties are shown in
Figure 6. The unmodified MMT control shows that without
any surface modifier, the hydrophilic clay is incompatible
with the ionomer resulting in a micron-scale
dispersion (opaque bars with visible particles) and almost
no reinforcement (very low effective aspect ratio). While
the modulus increase trends are similar to the sodium
ionomer system, the reinforcement was significantly
higher for all compositions. Since the melt viscosity of
the zinc and sodium ionomers have similar rheological
properties, the data indicate that the divalent zinc Surlyn
was more thermodynamic compatible with the M
2(HT)
2
modified clay, resulting in a superior morphology. This
finding is consistent with the results reported by Paul [4-
6]. As with the sodium ionomer, while the excess
M
2(HT)
2 modifier increased the inter-gallery spacing (to
reduce the cohesive force), it did not make a measurable
change to the tensile properties.
As shown in Figure 12, the more polar MH(HT)
2
modified Cloisite 93A produced a partially exfoliated
micro/nano structure that was inferior to that of the Cloisite
20A sample. However, the dispersion quality of the
zinc ionomer composite was far superior to that of the
sodium ionomer system. The tensile data for both sodium
and zinc ionomer nanocomposites are summarized in
Figures 7. Zinc ionomer nanocomposites had significantly
higher modulus over the entire composition space
tested, but had lower toughness (energy to break) for
most states. While it cannot yet be established why the
zinc system produced a marked improvement over the
sodium ionomer in dispersion and tensile properties, it is
postulated that the zinc ionomer matrix was thermodynamically
more compatible with both the M
2(HT)
2 and
MH(HT)
2 surface modifiers. The effects of the ions on
dispersion and surface interactions will be investigated in
future research.
In the last experiment, several grades of M
2(HT)
2
modified Laponite synthetic Hectorite and Fluorohectorite
layered silicates provided by Southern Clay Products
were melt compounded into the zinc ionomer. Figure 13
shows TEM micrographs of a Laponite B Fluorohectorite
composite with 5 wt. % clay content. Southern Clay
Products claims that the platelet diameter is on the order
of 25nm with a cation exchange capacity of 100
meq/100gm clay. WAXS measurements showed that the
inter-gallery spacing was approximately 42Å, the largest
of all silicates tested. This spacing was expected to reduce
the platelet cohesive force substantially, increasing
the likelihood of delamination in a polymer melt [1].
However, the dispersion was very poor, with much of the
clay bound in large micron and sub-micron sized agglomerate
particles. There were very few platelets found in
the TEM micrographs and a sparse population of small
tactoids was observed. Laponite RD Hectorite (25nm
platelets, CEC of 75 meq) produced a very poor micronscale
agglomerate dispersion as well. The bad dispersion
made it difficult to produce useful TEM micrographs.
Modified Laponite OG Hectorite (80 to 100nm platelets,
CEC of 75meq) was compounded into the zinc ionomer
with a resulting micro/nano-structure shown in the TEM
micrographs of Figure 14. The TEM micrographs indicate
that the dispersion was much better than that of both
25nm Laponite grades, but it was not as good as the 140
to 160nm platelet (presumed average sizes) Cloisite
MMT composites.
The tensile properties of the Laponite composites are
shown in Figure 6. The reinforcement with clay type and
loading was consistent with the extent of exfoliation
achieved during compounding and the silicate platelet
size estimates. While the overall structure and surface
charge mechanisms are similar for Hectorite, Fluorohectorite
and MMT, slight surface charge distribution and
chemistry differences may have affected dispersion kinematics
and thermodynamic interactions. If one assumes
that the van der Waals and ionic interactions were
similar, then the test results indicate that platelet dimensions
influence melt compounding dispersion dynamics.
These results would confirm Kamal’s work showing how
large lateral dimensions of platelets reduce the hydrodynamic
stress required for breakup to occur in polymer
melts [1, 9].
The structure-property relationships for the zinc ionomer
nanocomposites were examined using composites
theory [3-6]. The Halpin-Tsai equations were used to
predict the Young’s modulus and estimate an effective
aspect ratio of the layered silicate dispersions as list in
Table 1. Assuming good interfacial adhesion between the
matrix and filler, the aspect ratios are consistent with the
structures shown in the TEM micrographs and the tensile
reinforcement. Unmodified MMT clay produced a micron-scale
dispersion providing little reinforcement with
an effective aspect ratio estimate, Af , of 5. The Cloisite
93A [MH(HT)
2] composites had an Af of 18, approximately
half that of the M
2(HT)
2 modified MMT samples.
Conclusions
Melt compounding experiments were conducted on a
twin screw extruder to disperse organically modified
layered silicates into sodium and zinc ionomers. The
results identified key factors that govern the exfoliation
process in a polymer melt under shear that create the
morphology and subsequently influence physical properties.
These key factors include:
1) For the Surlyn ionomers tested, an appropriate organic
modifier is required to provide thermodynamic compatibility in the melt state to promote a higher
extent of exfoliation or tactoid size reduction while
maximizing the effective aspect ratio of the particles.
2) Ethylene copolymers neutralized with different cations
(sodium and zinc in these experiments) can alter
the compatibility between the polymer and the
modifier, resulting in significant changes to the morphology
and tensile properties.
3) The stress generated during melt compounding does
affect exfoliation and tactoid size reduction. On a
laboratory extruder, barrel temperatures can be used
effectively to change the melt viscosity and move the
melt mixing zone from high to low stress states.
4) Layered silicate geometry (platelet size or aspect
ratio) impacts the extent of exfoliation and tactoid
size reduction. Layered silicates with large lateral
platelet dimensions, like Montmorillonite, may be
more easily dispersed in a polymer melt provided the
components have good thermodynamic compatibility.
Further research is required to develop an understanding
of the roles that the ionomer cations play in the thermodynamic
interactions between the filler, modifier and
polymer.
Acknowledgements
The author is grateful to Southern Clay Products
(SCP) who produced the organically modified clays. The
author acknowledges the advice and stimulating conversations
with Doug Hunter at SCP and Professor Don Paul
at the University of Texas at Austin.
References
1) S. N. Bhattacharya, R. K. Gupta, M. R. Kamal, “Polymeric
Nanocomposites, Theory and Practice,”
Hanser Gardner, Cincinnati, 2008, 35-104.
2) D. L. Hunter, K. W. Kamena, D. R. Paul, MRS Bulletin,
Volume 32, April 2007, 323-327.
3) T. D. Fornes, D. R. Paul, Polymer 44 (2003) 4993–
5013.
4) R. K. Shah, D. R. Paul, Polymer 46, 2005, 2646–
2662.
5) R. K. Shah, D. R. Paul, Macromolecules, 39, 2006,
3327-3336.
6) L. Cui, C. Troeltzsch, P. J. Yoon, D. R. Paul, Macromolecules,
March, 2009.
7) J. Gao, G. Walsh, D. Bigio, R. Briber, M. Wetzel,
AIChE Journal, 1999, 45, 2541-2549.
8) J. Gao, G. Walsh, D. Bigio, M. Wetzel, Polymer Engineering
and Science, 40, 2000, 227-237.
9) N. Borse, M. Kamal, Polymer Engineering and
Science, 49, 4, 2009, 641-650.
10) K. Wang, L. Chen, J. Wu, M. L. Toh, C. He, A. F. Yee, Macromolecules, 38, 2005, 788-800
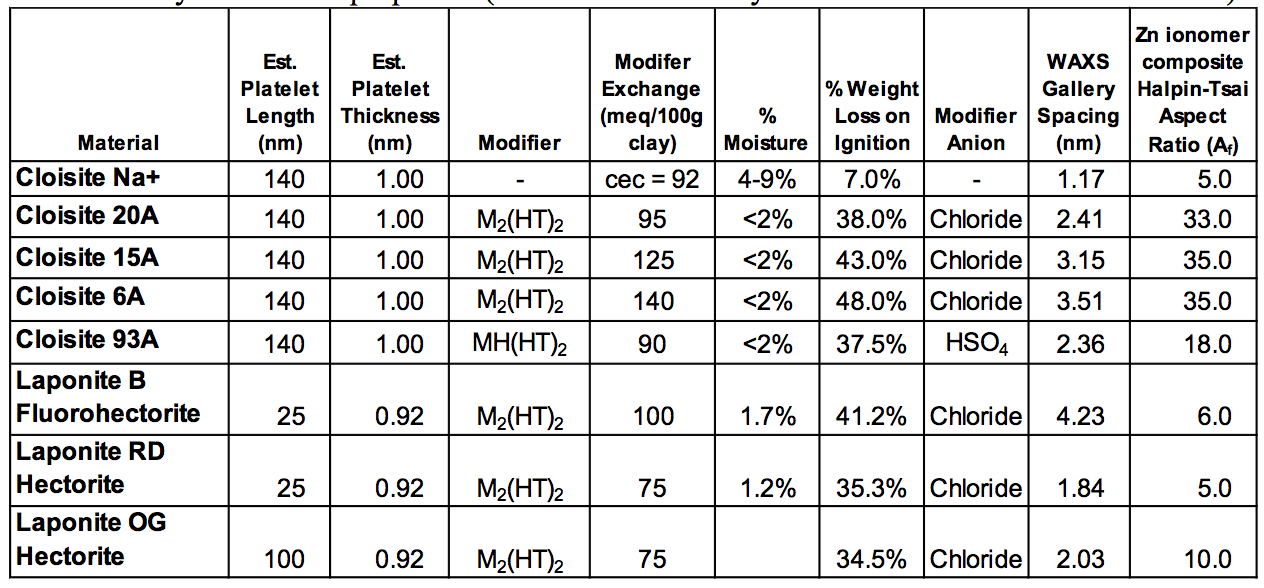
Table 1. Layered silicate properties (source Southern Clay Products and WAXS measurements).
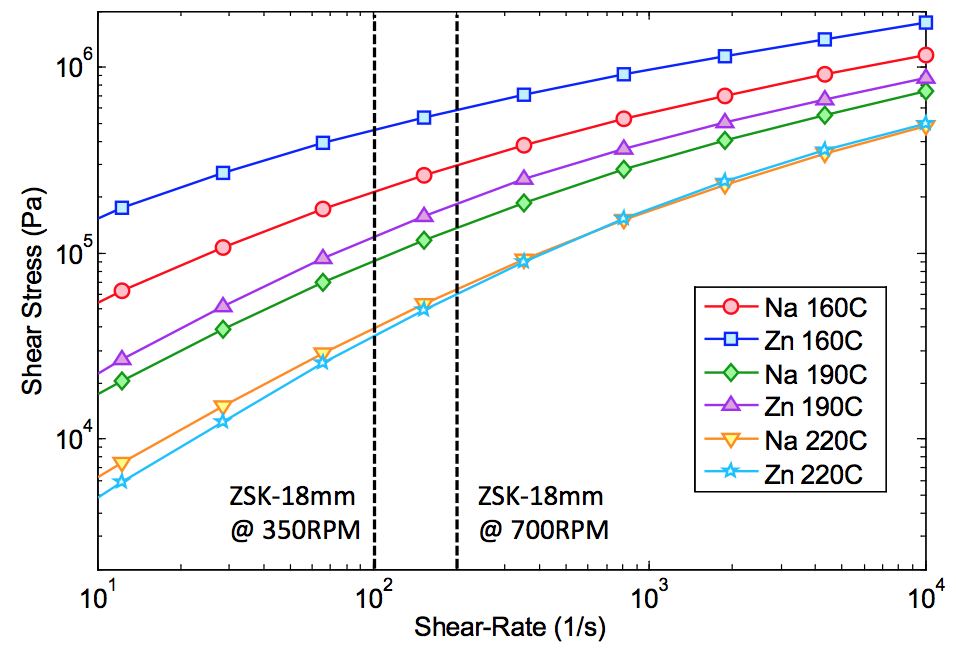
Figure 1. Sodium and zinc ionomer shear stress vs. shear rate curves at different operating temperature regimes.
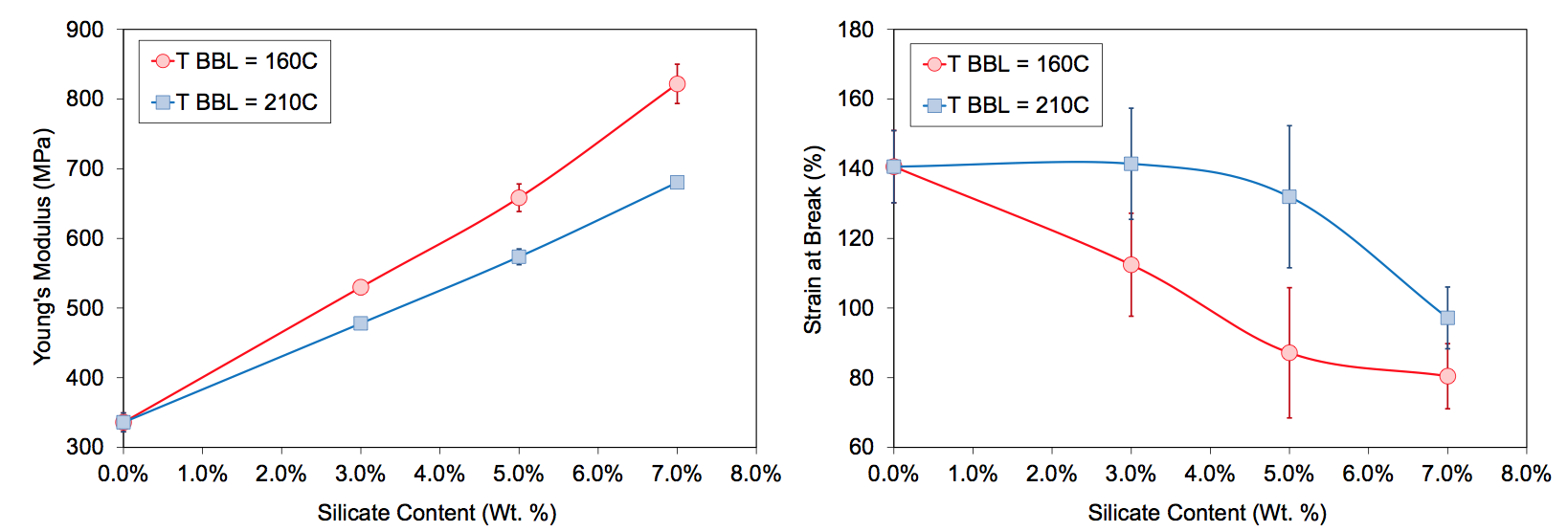
Figure 2. Tensile properties of injection molded bars of letdown extrusions of Sodium ionomer / Cloisite 20A master- batches made under low and high temperature conditions (error bars are ± one standard deviation, 6 bars per state).
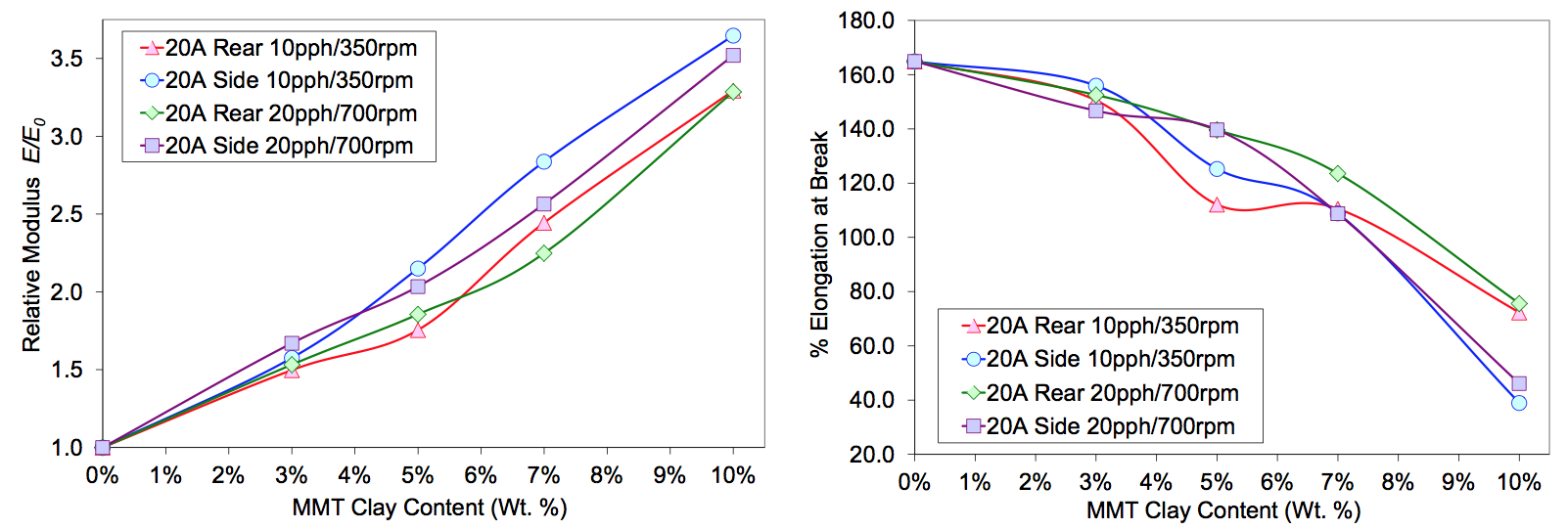
Figure 3. Tensile properties for constant Q/N samples run on a ZSK-18mm extruder with MMT fed in the rear or side ports.
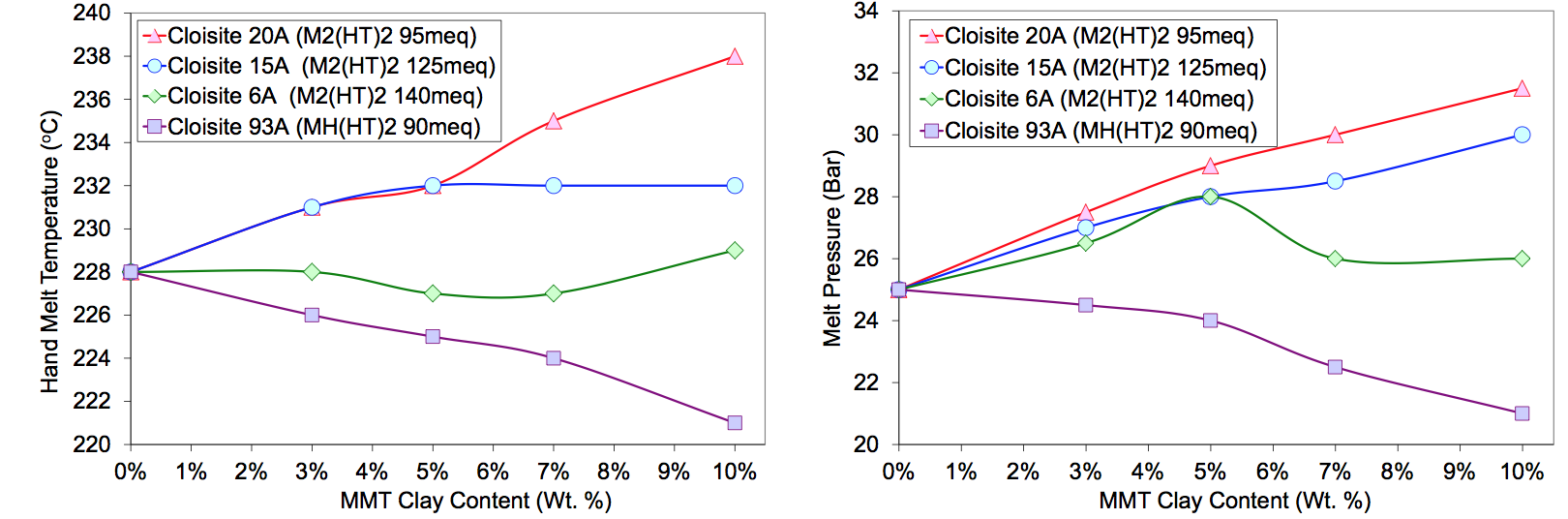
Figure 4. Sodium ionomer / organically modified MMT extrusion melt temperature and pressure.
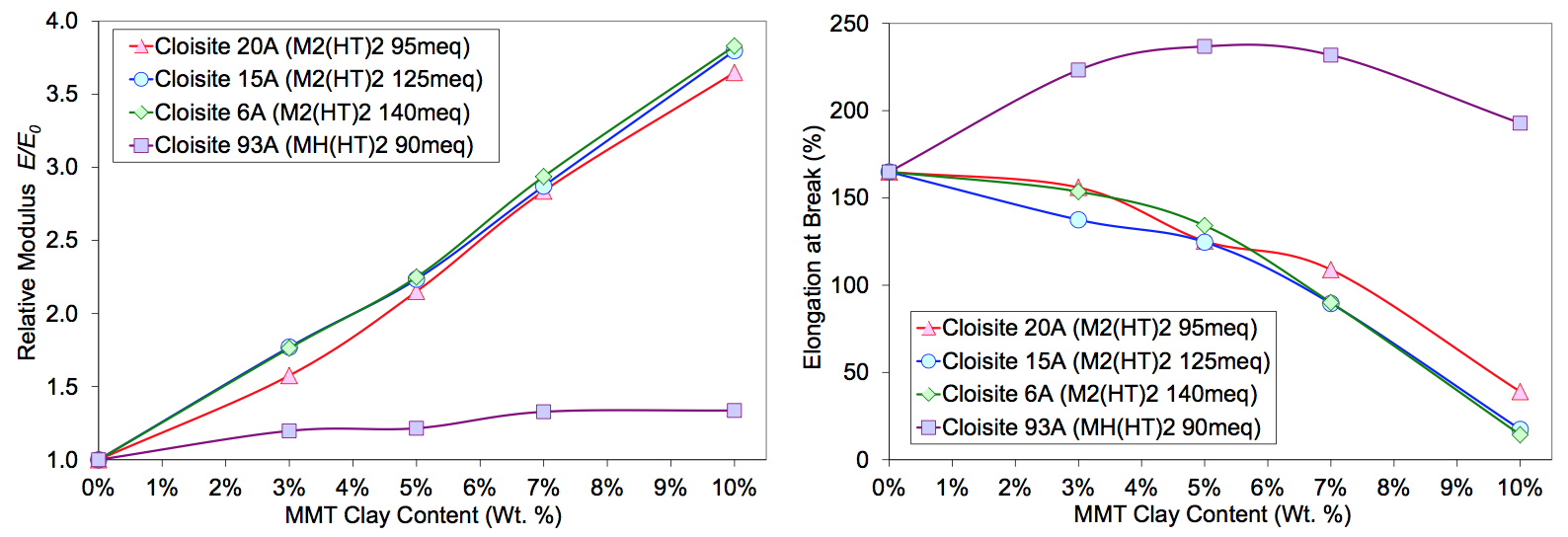
Figure 5. Sodium ionomer / organically modified MMT tensile properties.
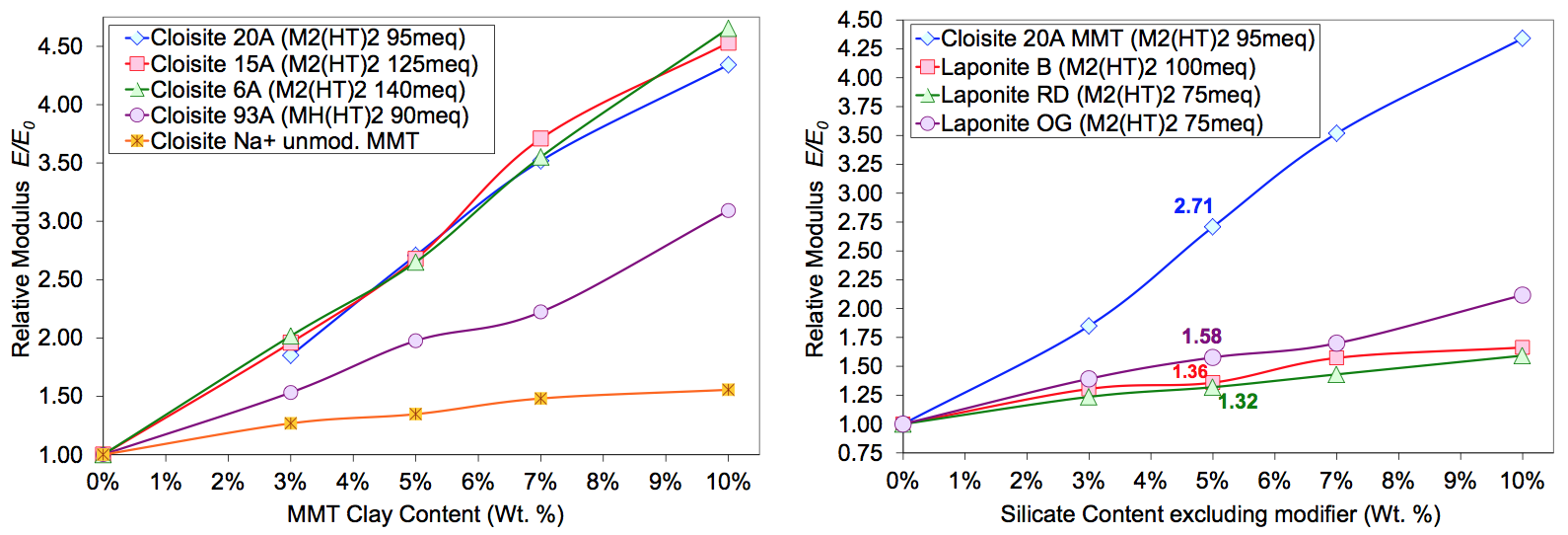
Figure 6. Tensile modulus data for zinc ionomer / layered silicate composites.
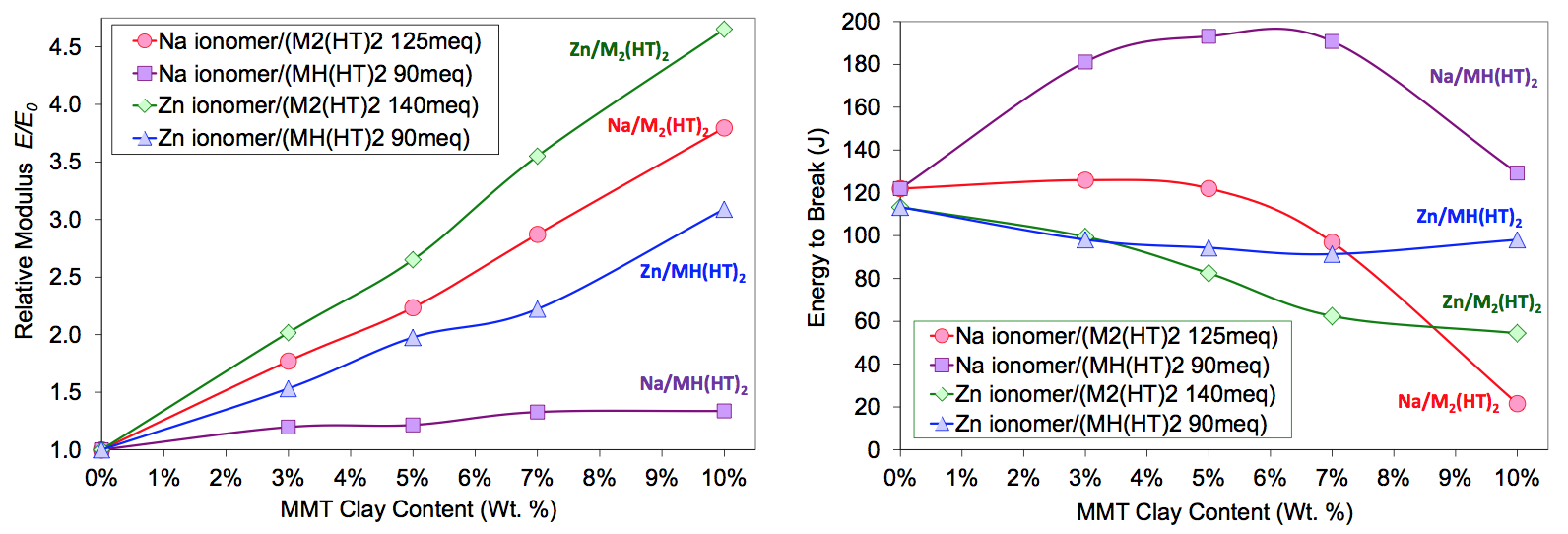
Figure 7. Tensile data comparing zinc and sodium ionomer / Cloisite MMT nanocomposites.
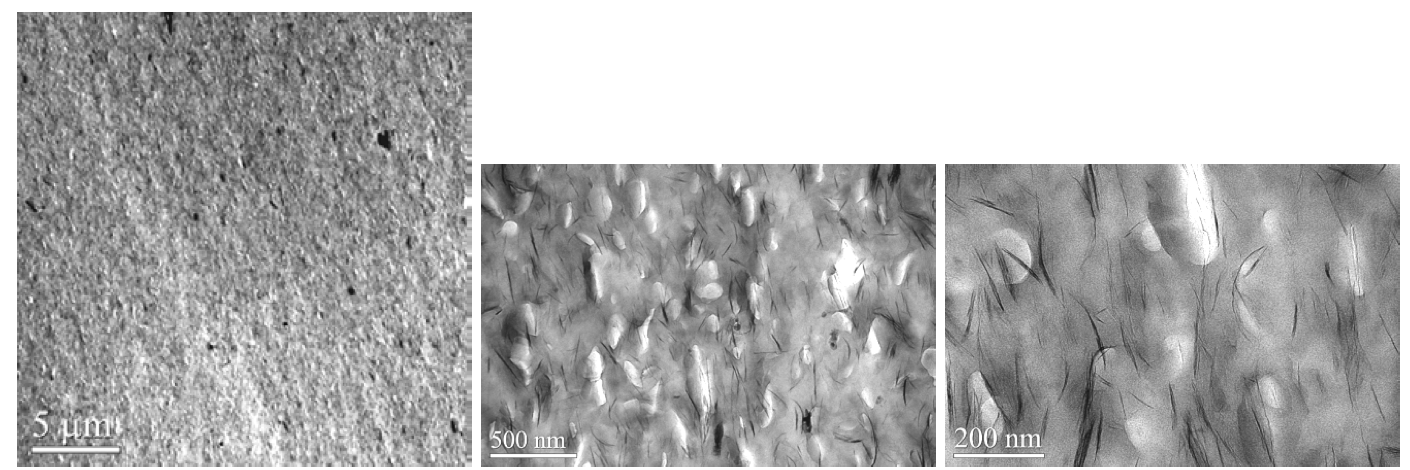
Figure 8. TEM images of sodium ionomer/Cloisite 20A (5 wt. % MMT) composite molded bar (10 lb/hr and 350RPM).
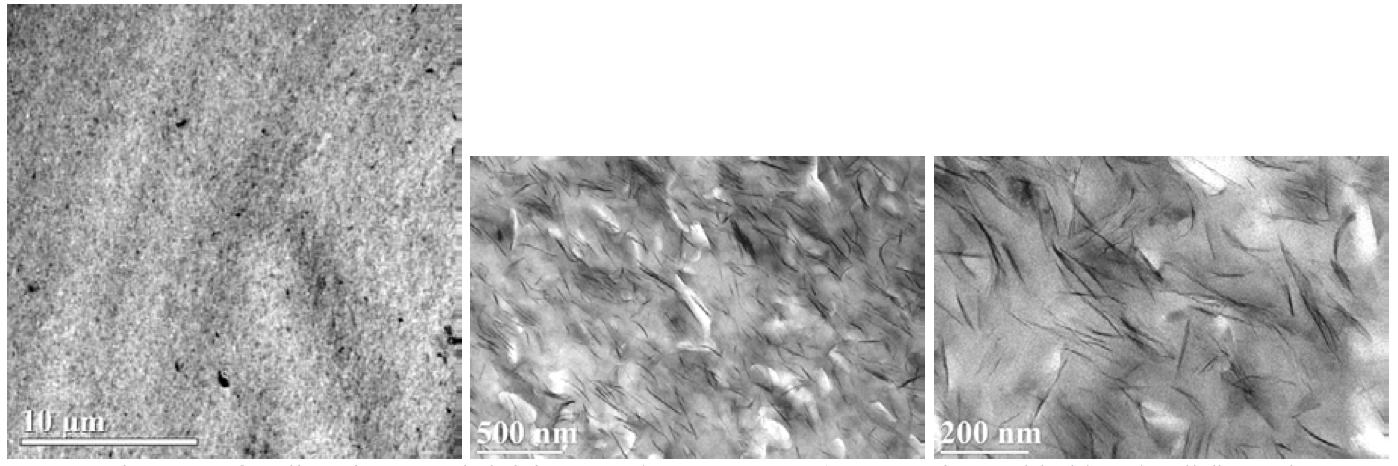
Figure 9. TEM images of sodium ionomer/Cloisite 15A (5 wt. % MMT) composite molded bar (10 lb/hr and 350RPM).
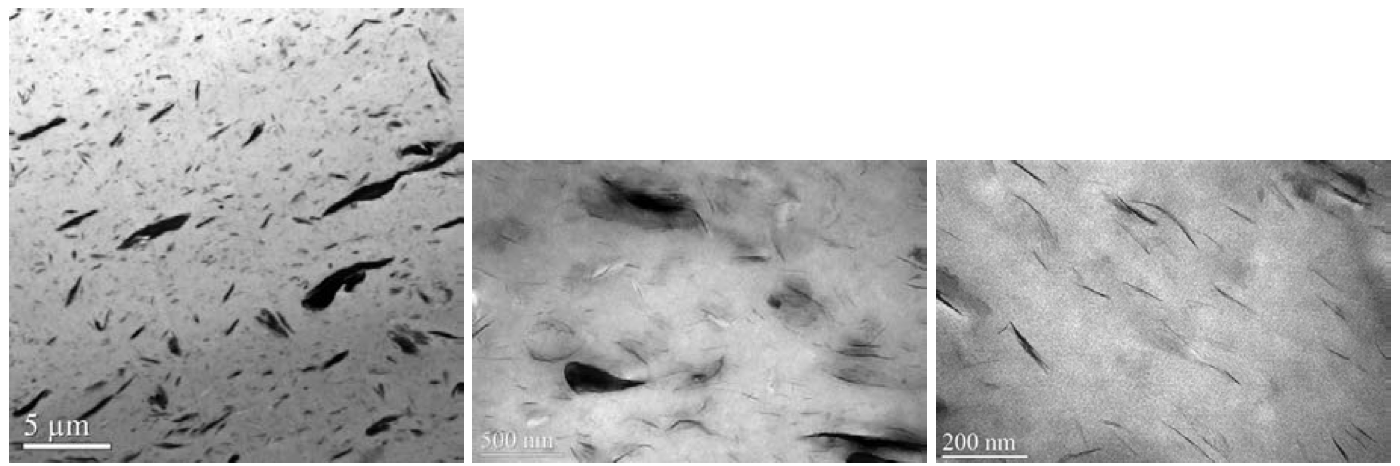
Figure 10. TEM images of sodium ionomer/Cloisite 93A (5 wt. % MMT) composite molded bar (10 lb/hr and 350RPM).
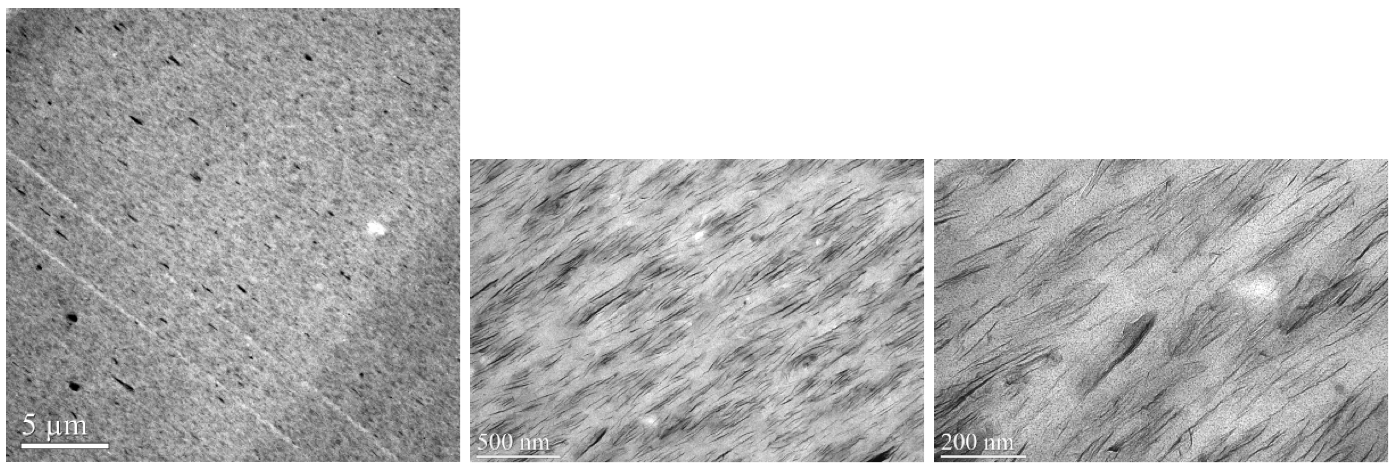
Figure 11. TEM images of zinc ionomer / Cloisite 20A (5 wt. % MMT).
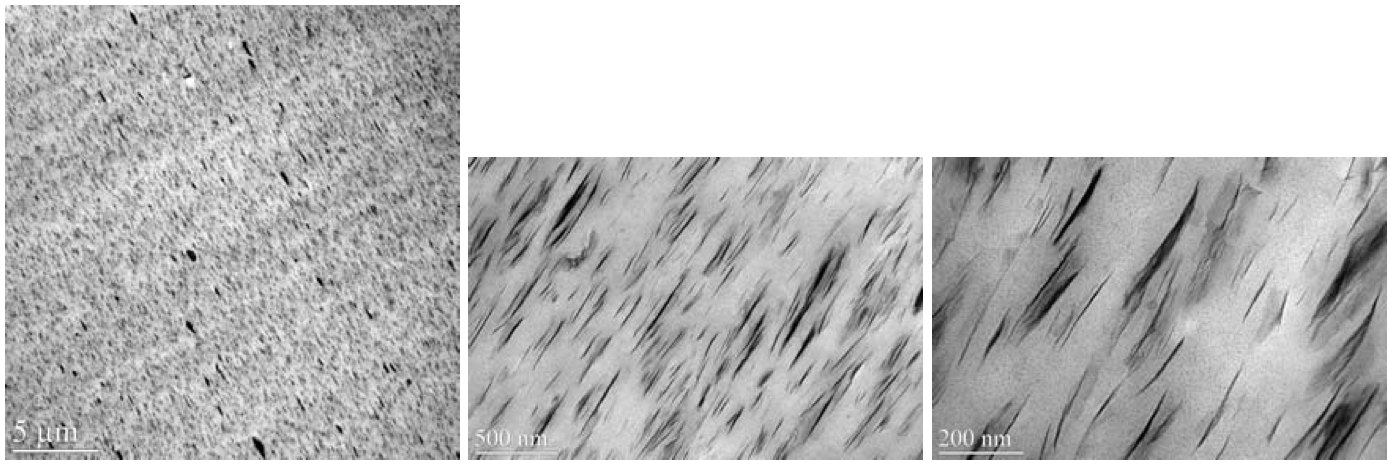
Figure 12. TEM images of zinc ionomer / Cloisite 93A (5 wt. % MMT).
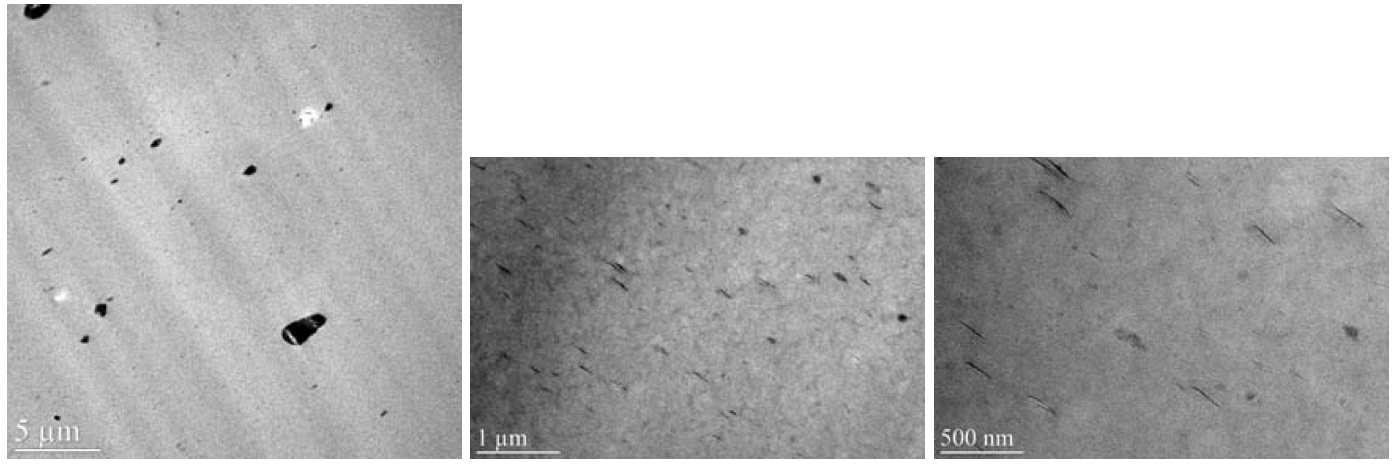
Figure 13. Zinc ionomer / organically modified Laponite B Fluorohectorite composite (5 wt. % clay) TEM.
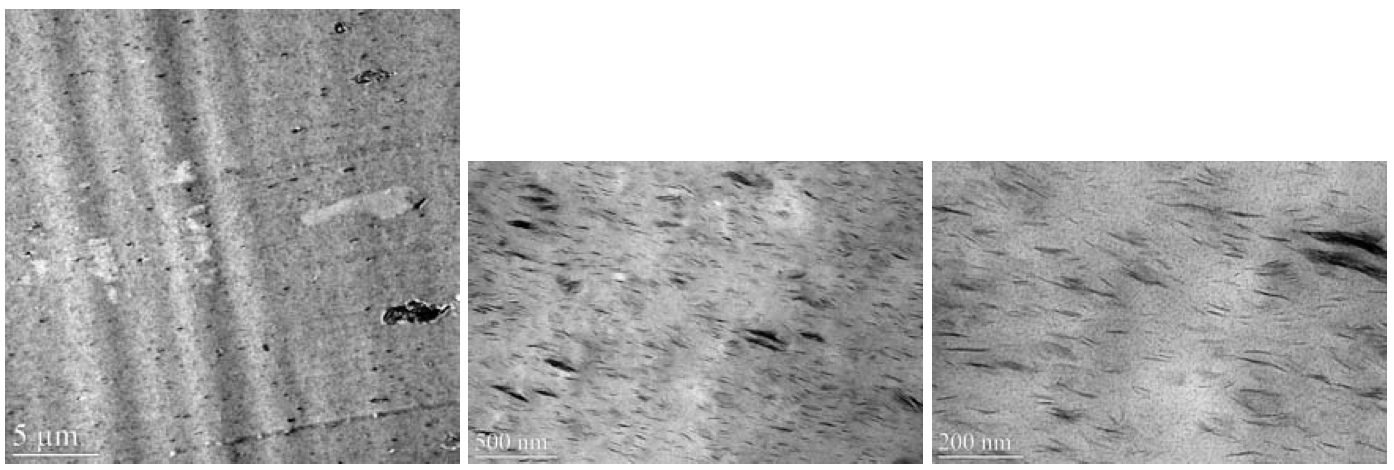
Figure 14. Zinc ionomer / organically modified Laponite OG composites (5 wt. % clay) TEM.
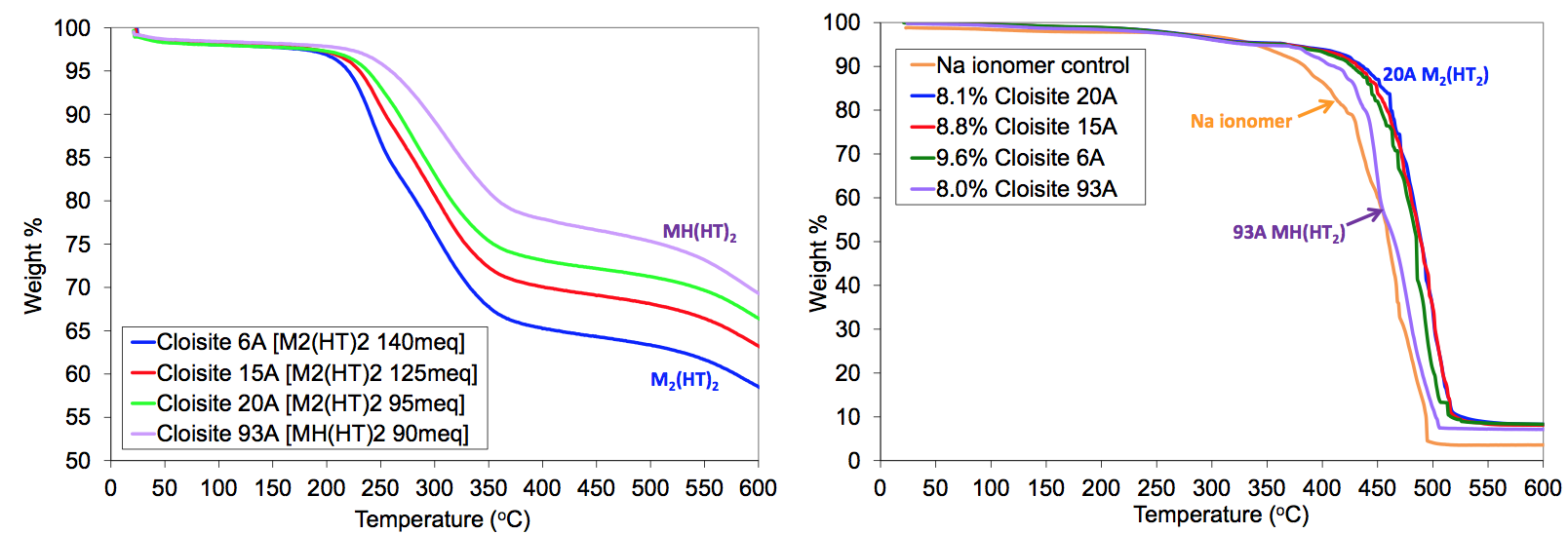
Figure 15. Thermogravimetric analysis (TGA) of organically modified MMT and sodium ionomer nanocomposites in air.
The advice contained herein is based upon tests and information believed to be reliable, but users should not rely upon it absolutely for specific applications since performance properties will vary with processing conditions. It is given and accepted at user’s risk and confirmation of its validity and suitability in particular cases should be obtained independently. The DuPont Company makes no guarantees of results and assumes no obligation or liability in connection with its advice. This publication is not to be taken as a license to operate under, or recommendation to infringe, any patents.
Return to
Paper of the Month.