Flex Barrier Property Enhancement in Film Structures Using Microlayer Coextrusion Technology
Patrick C. Lee, Joseph Dooley, Jeff Robacki, Steve Jenkins, and Robert Wrisley
The Dow Chemical Company, Midland, MIAbstract
Microlayer coextrusion is a process in which two or
more polymers are extruded and joined together in a
feedblock or die to form a single structure with multiple
layers. This paper describes a multilayer coextrusion
process technology to maintain the gas barrier property of
Ethylene Vinyl Alcohol (EVOH) barrier films after
flexing.
Introduction
Coextrusion is a common method used for producing
multilayer cast and blown films. Coextrusion is a process
in which two or more polymers are extruded and joined
together in a feedblock or die to form a single structure
with multiple layers. This technique allows the processor
to combine the desirable properties of multiple polymers
into one structure with enhanced performance
characteristics. The coextrusion process has been widely
used to produce multilayer sheet, blown film, cast film,
tubing, wire coating, and profiles [1-6].
Currently films containing metal foil dominate the
barrier packaging market with over 15.4 billion dollars in
global laminate film sales. Despite the dominance of the
foil films which provide excellent unflexed barrier
performance at a low price, alternative packaging solutions
that maintain barrier performance after flexing, amenable
to microwave operation, transparent enough to view the
packaged contents inside, and offer sustainability and a
lower carbon footprint are highly desirable. Currently, no
clear polymer can offer the oxygen barrier performance of
foil, in a low carbon footprint, competitive cost solution.
Ethylene Vinyl Alcohol (EVOH) comes the closest to
matching the oxygen, clarity and cost parameters.
However, flex barrier properties of EVOH barrier films are
poor due to the brittle nature of EVOH. EVOH barrier
packaging products with an improved flex barrier property
may provide an alternative packaging solution in foil
dominating liquid packaging markets.
Background
Attempts to maintain flex barrier properties until now
have been focused on using metalized layer lamination,
coating and/or inorganic particle addition in multilayer
structures with seven or fewer layers [7-14]. Recently, G.
Medlock and M. Dolgovskij studied EVOH flex crack
resistance between conventional EVOH films vs.
microlayer EVOH films [15]. Their study demonstrated
that microlayer EVOH films yielded superior flex crack
resistance compared to films with a single EVOH layer. In
addition, they claimed that lower modulus polymers such
as PE based ties compared to Nylon resist crack
propagation from one EVOH microlayer to another. The
number of layers was varied from 7 to 21 layers and the
sample went though up to 1,000 Gelbo flexing cycles.
This study and G. Medlock and M. Dolgovskij’ study
using microlayer technology clearly have advantages over
previous art by (i) improving recyclability due to the use of
polymer-only systems (i.e., no inorganic/metallic
particles), (ii) avoiding an extra step such as lamination, or
coating, or chemical vapor deposition, (iii) maintaining
transparency, and (iv) allowing microwaving. Furthermore,
this study covers larger number of layers up to 35 layers
with statistical analysis to confirm the barrier property
enhancement with respect to the number of layers.
Experimental
Barrier and tie materials used in this study are listed in
Table 1. The barrier resins of choice were EVAL L171 and
H171 grades from Kuraray America Inc. The % mol
ethylene content, Melt Flow Index, and density
information are listed in Table 1. Tie materials were blends
of Dow AMPLIFY (Trademark of The Dow Chemical
Company) and AFFINITY resins to vary the density from
0.875 to 0.9 g/cc. The skin material was Dow Low Density
Polyethylene (LDPE 503A) resin. This resin has a Melt
Flow Index of 1.9 g/10 min (190
oC/2.16 kg). The core to
skin ratio of the film structure was set to 20:80. The overall
film thickness was approximately 127 micrometers (5
mils). The design of experiment (DOE) was created using
4 independent variables: number of layers, barrier resin
type (% mol ethylene), tie material density, and EVOH/Tie
ratio in the core. The detailed DOE is shown in Table 2.
The coextrusion line used in this study consists of
three 38.1 mm (1.5 inch) diameter, 30:1 L/D or 24:1 L/D
single screw extruders. Extruders A and B feed a three
layer feedblock coupled with layer multipliers (see Figure
1). A 5 layer structure was created by operating all three
extruders without a multiplier (3 core layers+2 skin layers).
A four and a two channel multiplier were used to create 19
layer samples (17 core layers+2 skin layers). 17 core layers
were calculated by counting double layers as one layer
after multiplications. For example, initial 3 layers become
12 layers after flowing through a four channel multiplier.
However, 3 internal mating layers during re-stacking process are A-A double layers (Figures 1 and 2).
Therefore, actual flow structure has 9 A-B alternating
layers. After this flow goes through a two channel
multiplier, it has 17 alternating layers (9 x 2 – 1 double
layer = 17 alternating layers). In a same way, two four
channel layer multipliers were used to create 35 layer
samples (33 core layers+2 skin layers). 33 core layers are
calculated as follows: 3 X 4 = 12 layers – 3 double layers =
9 alternating layers; 9 X 4 = 36 layers – 3 double layers =
33 alternating core layers).
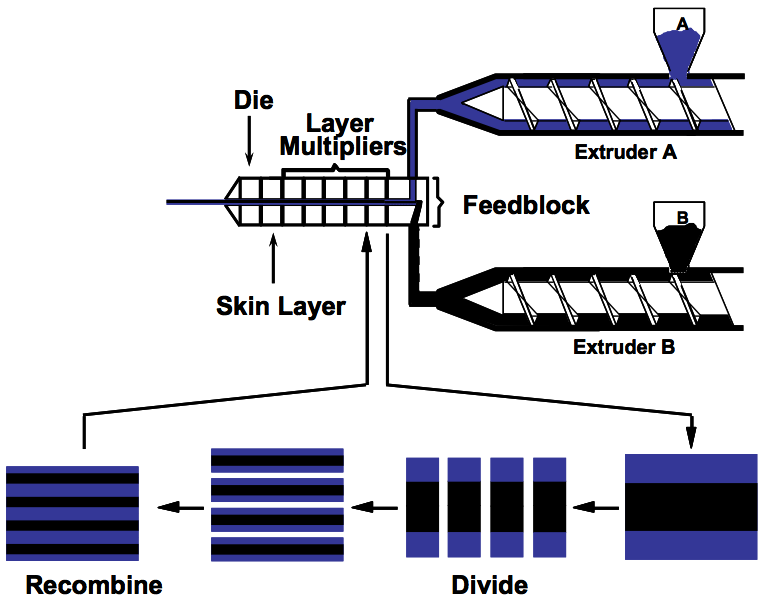
Figure 1. Coextrusion Line Set-up
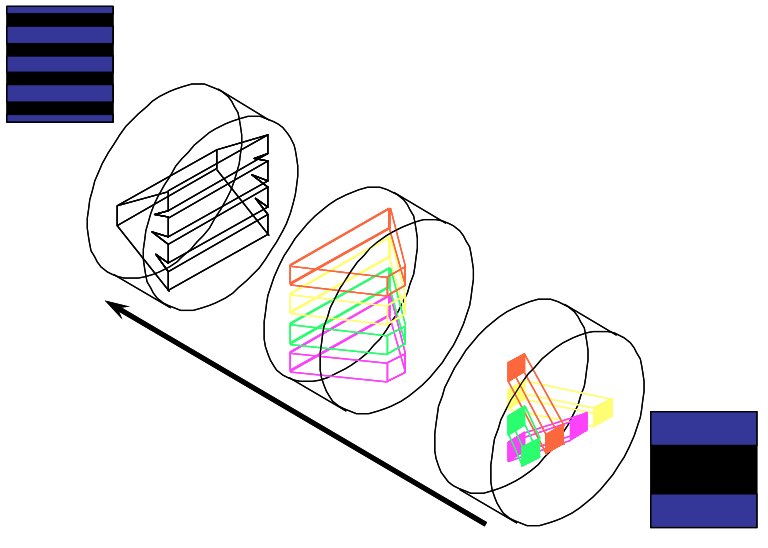
Figure 2 shows a schematic of the four channel layer multiplier. This multiplier creates 9 alternating layers from 3 layers as described earlier.
The multilayered structures are further sandwiched
with two skin layers by another extruder before entering a
304.8 mm (12 inch) coat hanger style die with a 0.559 mm
(0.022 inch) die lip gap.
The processing temperatures for all extruders,
feedblock, multipliers, and die were maintained at 210
oC
(410°F) except at the feeding zones of the extruders. The
microlayer samples were made of 20% of a core structure
containing the alternating microlayers, and 80% of a skin
structure equally divided between each side of the core.
The core material ratio was varied from 30 vol%/70 vol%
to 70 vol%/30 vol% in the core (see Table 2). The overall
flow rate was maintained at 27.2 kg/h (60 lb/h).
Film samples were tested for the following physical
properties using the ASTM standards listed in the Table 3.
Results
Effect of Flex Cycles on Oxygen Barrier Property
Oxygen transmission rate (OTR) was measured at
23
oC and 85% relative humidity before and after flexing
(400 Gelbo flex cycles) on the samples with fewer than 2
pin holes in the structure. The compositions and layer
numbers of these films are shown in Table 4.
OTR measurements for the sample 17 with 5 layers
before and after flexing were included to demonstrate the
detrimental effect of flex cycles on the samples with a
small number of layers (Table 4). After 400 flex cycles, the
5 layer sample failed the OTR test. The permeation value
of 65 cc-mil/100in
2.day is the maximum measureable
number by the Dow MOCON unit and is shown in
parentheses for the reference.
The OTR measurements on the four higher number of
layer samples (sample #: 5, 7, 9, & 11) demonstrate that
the flex barrier property was maintained after 400 Gelbo
flexes (Table 3). % OTR change before and after flexing
with respect to the number of layers is shown in Figure 3.
The samples 5 and 11 data were used for 19 and 35 layer
cases, respectively, and the permeation value of 65 ccmil/100in
2.day was used to calculate % OTR change for
the 5 layer case. As depicted in Figure 3, the 19 and 35
layer samples maintained the flex barrier property after
flexing but the 5 layer sample lost the barrier property. The
OTR measurement data on the samples 5 (19 layers) and
11 (35 layers) before and after flex cycles are shown in
Figures 4 and 5, respectively.
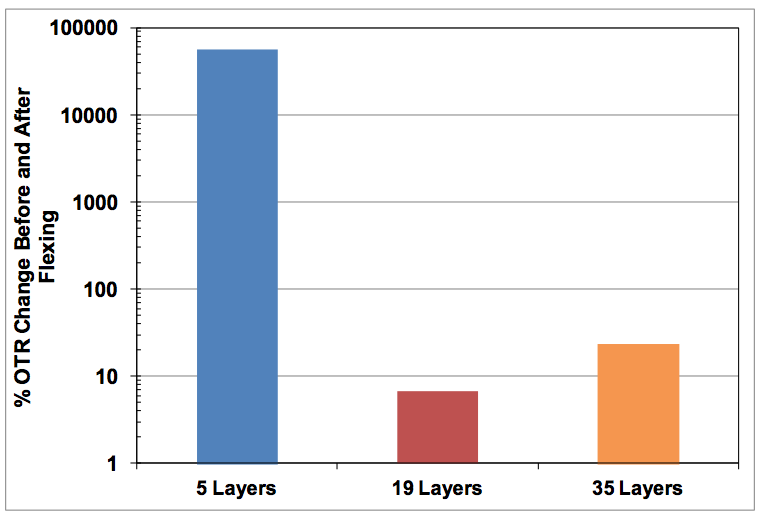
Figure 3. % OTR Change Before and After Flex
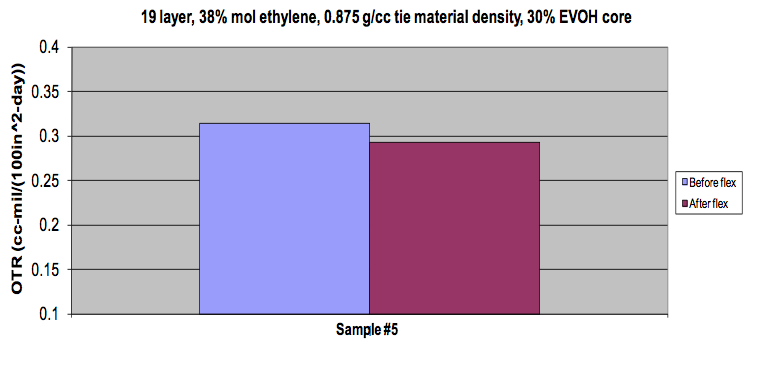
Figure 4. OTR Data of Sample 5 (19 layers) Before and After Flex Cycles: (a) Before and (b) After
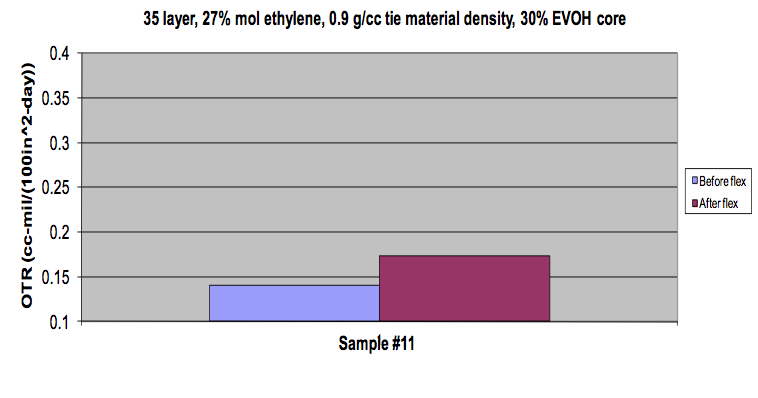
Figure 5. OTR Data of Sample 11 (35 layers) Before and After Flex Cycles: (a) Before and (b) After Flex
The AFM images before and after flex cycles, as
shown in Figures 6 and 7, were taken to demonstrate that
most of the EVOH (light layers) survived after flexing for
both 19 and 35 layer samples.
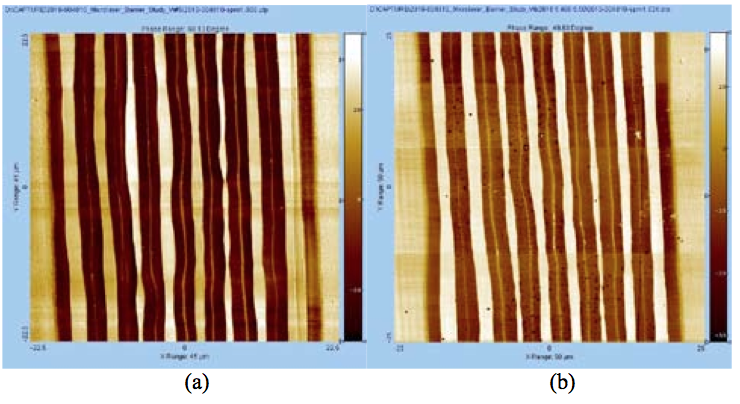
Figure 6. AFM Images of Sample 5 (19 layers) Before and After Flex Cycles: (a) Before and (b) After
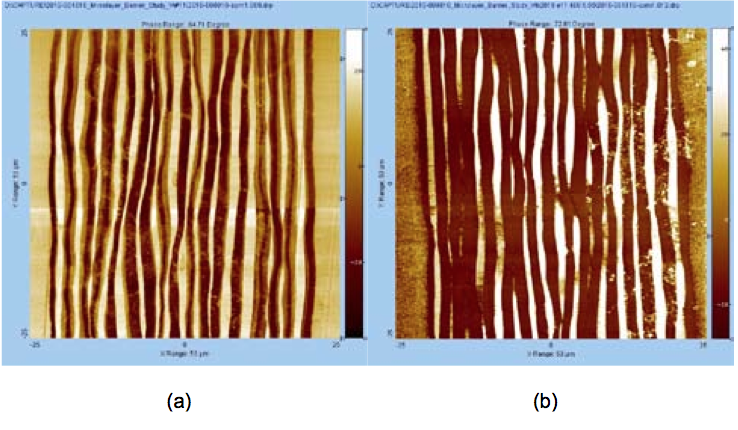
Figure 7. AFM Images of Sample 11 (35 layers) Before and After Flex Cycles: (a) Before and (b) After Flex
Effect of Layer Number on Pin Holes
Flex durability of our samples has been tested
according to ASTM F392-93. The flexing action consists
of a twisting motion followed by a horizontal motion, thus,
repeatedly twisting and crushing the film. After 400 cycles
of Gelbo flexes, physical holes through the structure were
measured by a color dye penetration method and the
average numbers of pin holes with respect to the number of
layers are shown in Figure 8.
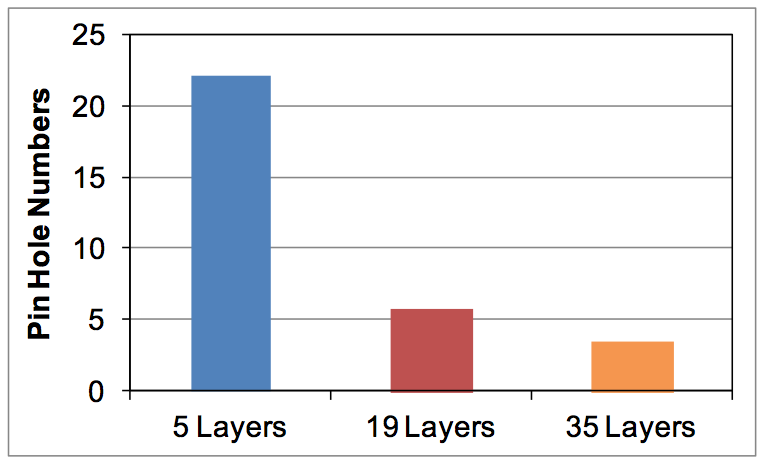
Figure 8. Pin Hole Numbers with respect to Number of Layers
The data was analyzed with a commercially available
statistical software (JMP, SAS institute Inc.). Figure 9
clearly demonstrates that the pin hole numbers statistically
decrease with the number of layers (a Tukey-Kramer test
with confidence = 95%). This is possibly due to: (i) better
flexibility of a brittle EVOH barrier resin in thinner layers
[16], and (ii) longer tortuous path for the dye to travel
through even if some layers were broken in 19 and 35 layer
samples [17, 18].
The effect of individual layer thickness of
Polyethylene terephthalate (PET) and Polycarbonate (PC)
microlayer films on large strain deformation mechanism
was studied earlier [16]. As the thickness of individual
polymer layers decreases, a transition in deformation
mechanism from two-component behavior to onecomponent-like
behavior was observed. Therefore, it is
possible to improve the film toughness by decreasing
EVOH layer thickness in the EVOH/PE based tie material
microlayer system.
A Cussler-Fredrickson model for permeation of small
molecules through polymer films filled with impervious
particles or crystalline lamellae describes that high content
of impermeable particles or crystalline lamellae, high
aspect ratio, and high angle of particles/lamellae to the
permeation direction (i.e., more perpendicular to the
permeation direction) gives less overall permeation of gas
molecules. For the EVOH microlayer case, EVOH can be
viewed as particles/crystals in the model to decrease the
overall permeation due to longer tortuous path [17, 18].
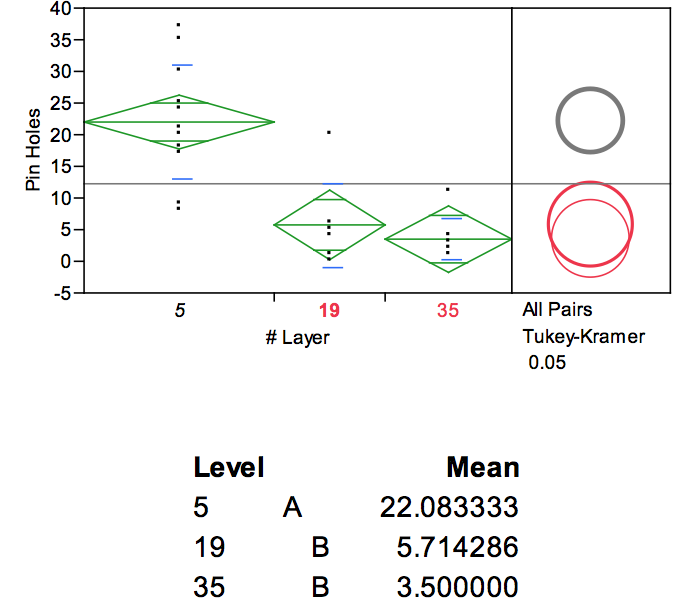
Figure 9. Effect of Layer Number on Pin Hole Numbers
Effect of Barrier Resin Type and Content
The extensive statistical analysis was performed to see
the effect of barrier resin type (% mol ethylene) and
content on barrier and mechanical properties. The expected
trends were observed; better oxygen barrier property was
observed with the samples containing the 27% mol
ethylene content barrier resin. Increasing the barrier resin
content improved better the barrier property. Machine and
cross direction mechanical properties such as tensile
strength and yield stress increased with higher EVOH
contents in microlayer samples while ultimate elongation
decreased. The samples with the 27% mol ethylene
content barrier resin behaved stiffer compared to the ones
with 38% mol content.
Conclusions
This study describes a process technology to maintain
or improve gas barrier property of EVOH barrier films
after flexing. EVOH and MA-g-PE multi- and microlayer
samples with 5, 19, and 35 layers were produced in a
coextrusion line. Flexing was performed using a Gelbo
flexing tester (400 flexes). After flexing, pin hole tests
were performed on the film and only those with fewer than
2 pin holes were re-tested for OTR measurements. The
clear trend of pin hole numbers decreasing after 400 Gelbo
flexes was observed as the number of layers increased. The
OTR measurements on these films demonstrated that thin
EVOH barrier resin can improve flex crack resistance of
the film. This proves that for a given amount of barrier
resin, the flex barrier property can be increased
dramatically by microlayering. This flex barrier
improvement in microlayers is possibly due to: (i) better
flexibility of a brittle barrier resin in thinner layers, and (ii)
longer tortuous path for gas molecules to travel through the
structure even if there are some broken layers. In addition,
mechanical properties of these microlayer films were
measured and evaluated statistically to understand the
general trend.
References
1. L.M. Thomka and W.J. Schrenk, Modern Plastics, 49,
4, 62 (1972).
2. C.D. Han, J. Appl. Poly. Sci., 19, 7, 1875 (1975).
3. W.J. Schrenk, Plastics Engineering, 30, 3, 65 (1974).
4. J.A. Caton, British Plastics, 44, 3, 95 (1971).
5. L.M. Thomka, Plastics Engineering, 18, 2, 60 (1973).
6. C.R. Finch, Plastics Design Forum, 4, 6, 59 (1979).
7. T. D. Kendig, U.S. Patent 6,623,821 B1 (2003).
8. P. Frisk, U.S. Patent 5,916,685 A (1999).
9. K. Nakamura, U.S. Patent 20070160806 A1 (2007).
10. H. Umekawa and Y. Inui, U.S. Patent 20020197480
A1 (2002).
11. B. Bastion Bradle and R. Komro, U.S. Patent
20060046006 A1 (2006).
12. M. Yamazaki, H. Tanaka, and Y. Inaba, U.S. Patent
20070134476 A1 (2007).
13. K. Miharu, and H. Tachino, U.S. 6,011,115 A (2000).
14. K. P. Nelson and C. J. Harvey, U.S. Patent
20070031546 A1 (2007).
15. G. Medlock, and M. Dolgovskij, TAPPI PLACE
Conference (2012).
16. R. Adhikari, V. Seydewitz, K. Loeschner, G. H.
Michler, A. Hiltner, and E. Baer, Macromolecular
Symposia, 290, 156 (2010).
17. E.L. Cussler, S.E. Hughes, W.J. Ward, and R. Aris,
Journal of Membrane Science, 38, 161 (1998).
18. W.S. Jang, Ph.D. Dissertation, Mechanical
Engineering, Texas A&M (2008).
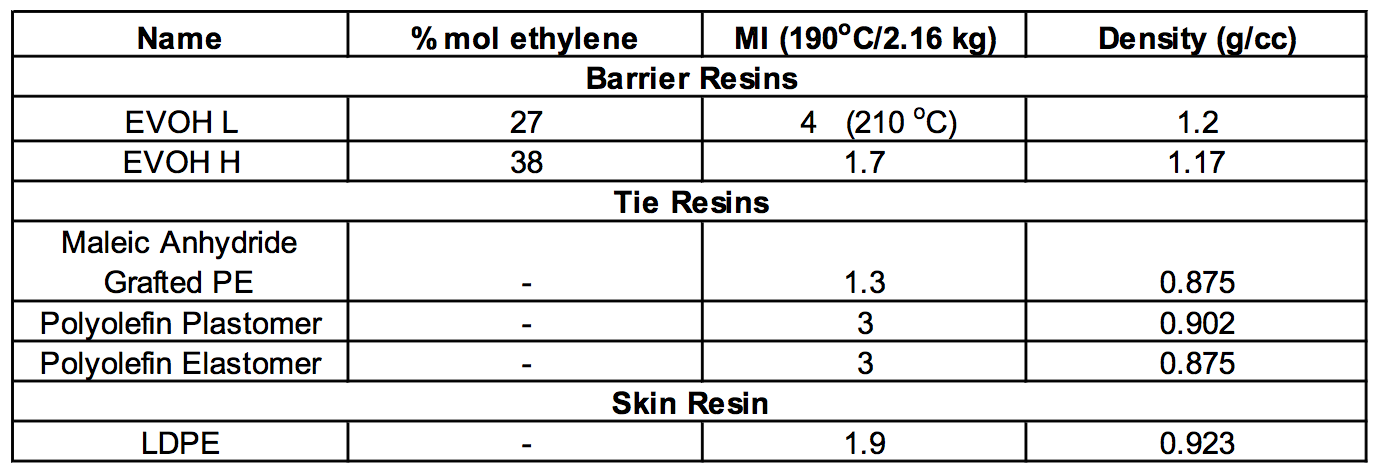
Table 1. Oxygen Barrier, Tie, and Skin Resin Properties
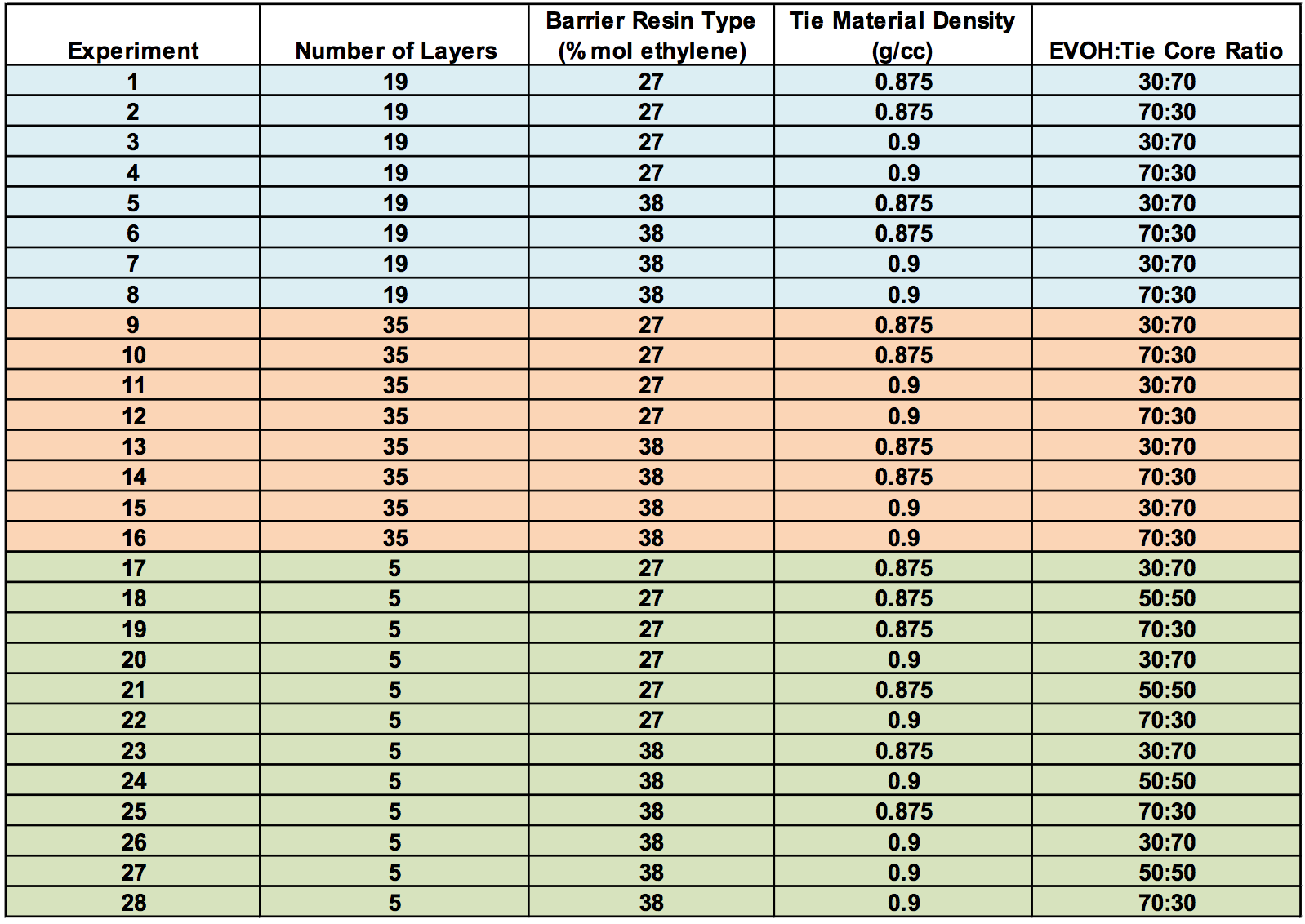
Table 2. DOE Table
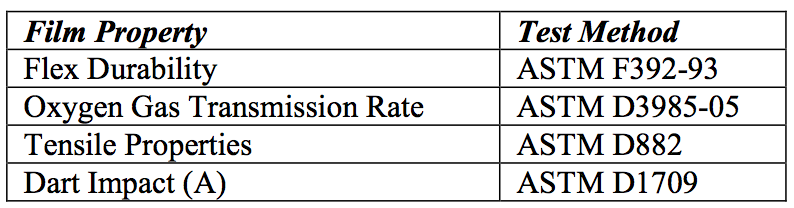
Table 3. Film Property Tests and Methods

Table 4. OTR Measurements Before and After 400 Gelbo Flexes
Return to
Paper of the Month.